What are gravitational waves?
Something a little different today, folks - lets talk about gravitational waves. What are they, where do they come from, and how can we measure them? The third detection of gravitational waves was recently confirmed by the LIGO team; indeed, the third such detection in 18 months! Exciting times for the entire scientific community!
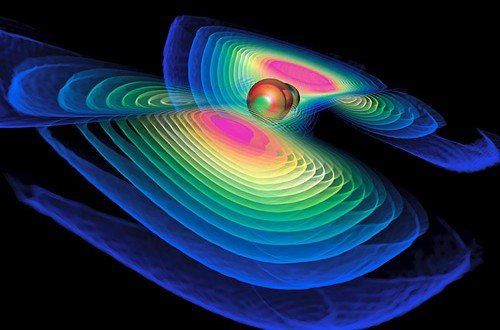
What are gravitational waves?
From general relativity, we know that mass produces spacetime curvature - this is demonstrated using the Earth as an example, in the figure below.
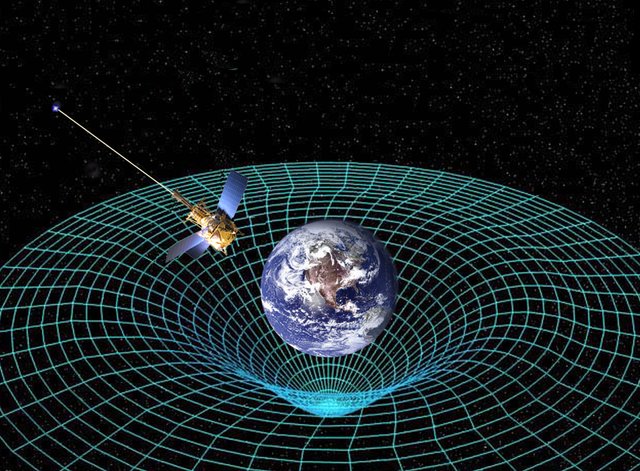
The laws of special relativity tell us that a finite speed limit is imposed on any type of possible communication. This constraint manifests as the well known speed of light, c.
Mass in motion gives rise to ripples in spacetime curvature that propagate at the speed of light: gravitational waves.
For the time being, we will just assume the existence of gravitational waves, and explore their properties. We will prove their existence mathematically in a future post.
Properties of gravitational waves
- They travel at the speed of light, c.
- They are transverse to the direction of propagation.
- They have two independent states of polarisation; namely, plus (+) and cross (x).
- They carry energy (in large quantities) away from a radiating system.
- They can be detected by considering their effects of freely-falling test bodies, or particles.
A linearised gravitational wave
The simplest example of a gravitational wave is a ripple in the curvature of space-time, propagating in one direction, independent of the other two (in 4-d spacetime):
- The direction of propagation (z) is the longitudinal direction.
- The other two directions (x and y) are transverse to the direction of propagation.
- It is a plane wave.
In order to deal with this concept mathematically (at first, simply), we will consider a spacetime geometry which is very close to being flat. We describe the geometry of spacetime via a quantity called the metric. We can have many different types of signature, which are useful in different contexts. In quantum field theory, in particular when dealing with the Dirac equation, or with a Yukawa potential for example, it is useful to use the signature (+, -, -, -). In this case, we will use the metric signature (-, +, +, +), which means the pure temporal and spatial components of the metric tensor are negative and positive, respectively. We can write the flat space metric in 4-d as a 4x4 matrix:
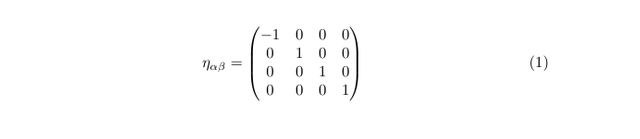
We are now able to treat the gravitational wave as a perturbation, which allows us to write a new metric in terms of the flat space metric, and some small deviation:

The metric is a tensor of rank 2. Don't worry too much about the details of this, we will revisit tensors in more detail in later posts on general relativity.
A simple example of a gravitational wave propagating along z is:
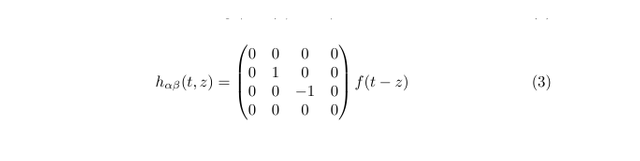
where we require |f(t-z)|<<1.
Typical amplitudes of |f(t-z)| are of the order 10^-21, so the linear approximation is not too shabby - we are only ignoring terms which are of order 10^-40.
Another import quantity is known as the line element of the metric. In some sense, we can think of this (informally) as a line segment which is associated with an infinitesimal displacement vector in a metric space. For the spacetime described by the perturbed metric we are considering, the line element can be written in the form:

The function f(t-z) encodes the size and shape of the gravitational wave, where f and h are dimensionless.
Examples:
- A gravitational wave of frequency omega, and amplitude a can be written:

- A Gaussian wave packet with width sigma, and amplitude b can be written:

Detecting gravitational waves
Spacetime curvature is detectable through the motion of test bodies. These are characterised as bodies which move along spacetime geodesics (paths which extremise proper time; solution of Euler Lagrange equations for path, dependent upon the square root of the metric) and produce no significant spacetime perturbation as they do so.
The detection of curvature requires monitoring the relative motion of two (or more) test bodies.
Now, lets see what happens to the motion of two test bodies, which are initially at rest, as the gravitational wave passes by. We need to consider their coordinate motion, as well as their proper distance.
It turns out that there is a fractional change in distance as the gravitational wave passes, which in the x direction, is given by:

where L_0 is the initial position of the test body relative to some reference point in the x direction. For a gravitational wave of amplitude a and angular frequency omega, we obtain

For a particle that is initially at a point L_0 in the y direction, we have the fractional change in distance

This result is easily generalised, and we can interpret delta(L)/L_0 as a fractional strain, which is produced by the gravitational wave.
Thanks for reading, in the next post on gravitational waves, we will consider the second polarisation, not covered in this post; namely, the cross (x) polarisation, and we will see where gravitational waves come from fundamentally as a solution to the Einstein field equations.
Interesting article
That went way over my head, do you have a dumb person version?
When massive objects move around (maybe two black holes orbiting eachother, for example), spacetime itself is changed. This "change" to the geometry of spacetime manifests as waveforms which propagate throughout the universe, and can be detected by measuring small changes in the distance of km scale laser beams, in the LIGO experiment (now advanced LIGO) . Hope this helps.
Reminded me of my college... Nice post though